The Reality of Quantum Computing: An In-Depth Analysis
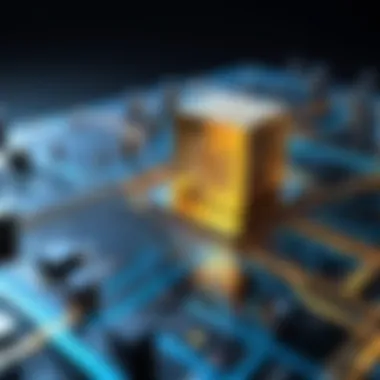

Intro
Quantum computing represents a significant shift in how we process information. Unlike traditional computers, which use bits as the smallest unit of data, quantum computers utilize quantum bits, or qubits. This fundamental difference allows quantum computers to perform complex calculations at unprecedented speeds.
The landscape of quantum computing poses various challenges and opportunities for businesses and researchers alike. Companies are investing heavily in this technology, aiming to leverage its potential for tasks that are presently impractical for classical computers. The goal of this article is to provide a thorough examination of quantum computing, from its basic principles to its far-reaching implications.
In the ensuing sections, we will highlight the key aspects of quantum computing technology, look at current advancements, and explore its potential applications across different sectors.
Prolusion to Quantum Computing
Quantum computing stands as a pivotal area of study within the realm of information technology. The convergence of physics, computer science, and engineering fundamentally redefines how we understand computation. Traditional computers process information in bits, expressed as 0s or 1s. In contrast, quantum computers leverage the peculiar properties of quantum mechanics, particularly superposition and entanglement, to operate on quantum bits or qubits. This shift not only enables potentially groundbreaking processing speeds but also opens pathways to solve complex problems considered infeasible by classical standards.
Defining Quantum Computing
Quantum computing encapsulates a novel approach to processing information, utilizing the principles of quantum mechanics. Unlike classical systems that rely on binary bits, qubits can exist in multiple states at once due to superposition. This unique characteristic permits operations on numerous possibilities simultaneously. As a result, quantum computers hold the potential to outperform classical machines in specific computational tasks, such as factoring large integers or simulating molecular structures.
Some of the key elements that define quantum computing include:
- Superposition: Allows qubits to represent both 0 and 1 simultaneously.
- Entanglement: A phenomenon where qubits become interconnected, such that the state of one qubit directly affects another, regardless of distance.
- Quantum Interference: Used to amplify correct answers while canceling out incorrect ones during computation.
Understanding these elements forms the cornerstone of grasping quantum computing's unique functioning and capabilities.
Historical Context
The voyage into quantum computing began in the early 1980s when physicist Richard Feynman proposed that traditional computers could not efficiently simulate quantum systems. This notion sparked interest, leading to theoretical frameworks that blended quantum mechanics with computer science. Notably, the 1990s marked a significant leap when Peter Shor developed an algorithm capable of factoring large numbers in polynomial time, underscoring the potential of quantum architectures in cryptography.
As investment in this technology increased, various organizations such as IBM, Google, and D-Wave began conducting practical experiments. This led to the development of prototypes and operational quantum systems by the 21st century. Institutions and corporations recognize that quantum computing promises transformative impacts across diverse sectors, hence the current surge in research and development.
As we delve deeper into the fundamentals of quantum computing, it is crucial to appreciate the foundational principles that guide this transformative technology. The implications it possesses extend far beyond academia, targeting industries and altering business paradigms.
Fundamentals of Quantum Mechanics
Understanding quantum mechanics is critical to grasp the underlying principles of quantum computing. Quantum mechanics is a branch of physics that explains how matter and energy behave at very small scales, such as atoms and subatomic particles. This topic is essential because it underpins the technology of quantum computing, providing a framework for how qubits operate and interact.
The significance of mastering quantum mechanics lies in its impact on computational speed and problem-solving capabilities. Unlike classical computers, which operate on bits that are either 0 or 1, quantum computers utilize quantum bits (qubits) that can exist in multiple states simultaneously. This characteristic results from the principles of quantum mechanics, making it possible for quantum computers to perform complex calculations much faster than traditional systems.
Key Principles of Quantum Mechanics
The key principles of quantum mechanics include superposition, entanglement, and wave-particle duality. Each concept plays a vital role in shaping the capabilities of quantum computing.
- Superposition allows qubits to represent both 0 and 1 simultaneously. This means a quantum computer can process a vast amount of possibilities at once, significantly increasing processing power.
- Entanglement enables qubits that are entangled to be correlated with one another, regardless of the distance separating them. Changes to one qubit will instantaneously affect its paired qubit. This property creates opportunities for enhanced data transmission and secure communication.
- Wave-particle duality illustrates that particles, like electrons, exhibit both wave-like and particle-like properties, merging into a duality that informs quantum computation processes.
These principles transcend traditional computational models, offering unique advantages in handling large-scale problems. For instance, they can revolutionize sectors like machine learning, optimizing algorithms far beyond classical capabilities.
Quantum Superposition and Entanglement
Quantum superposition and entanglement are cornerstone concepts that define the advantage of quantum computation over classical methods.
- Quantum Superposition: A qubit in superposition has the potential to perform several calculations at once. This feature alone allows quantum algorithms to solve complex problems, like factoring large numbers, in a fraction of the time needed by classical algorithms. This property is fundamentally useful in cryptography, where breaking encryption codes depends on the algorithm's ability to handle an immense number of calculations efficiently.
- Quantum Entanglement: This phenomenon provides quantum computers with an interconnectedness that classical systems lack. When qubits become entangled, the state of one qubit directly influences the state of another, creating a powerful network effect. This is especially beneficial for tasks requiring simultaneous data processing, such as simulations in physics and chemistry.
"Quantum entanglement challenges our conventional understanding of information transmission, paving the way for advancements in secure communications and teleportation concepts."
Quantum Bits: The Building Blocks
Quantum bits, or qubits, are fundamental entities in quantum computing. Their significance cannot be understated because they form the core of quantum information processing. Unlike classical bits, which are either 0 or 1, qubits can exist in multiple states simultaneously, thanks to quantum superposition. This unique property allows quantum computers to process vast amounts of data in parallel, offering an exponential increase in computational power for suitable algorithms.
Understanding Qubits
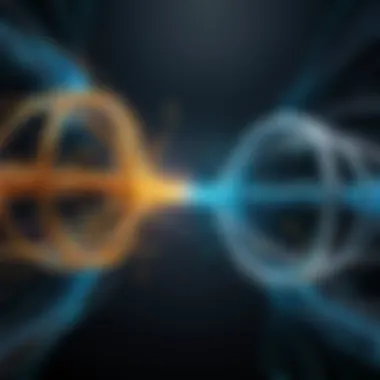
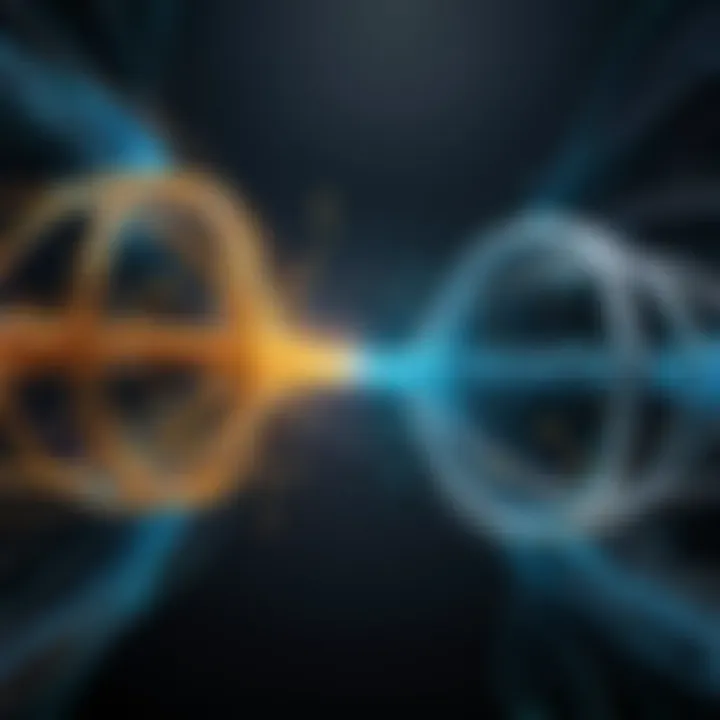
To fully grasp the role qubits play, it is essential to understand their inner workings. A qubit can exist in a state of 0, a state of 1, or any quantum superposition of these states. This ability to represent multiple values at once vastly enhances computational operations.
For practical purposes, physical qubits can be realized in various systems, such as:
- Superconducting Circuits: These qubits utilize the properties of superconducting materials.
- Trapped Ions: In this method, ions are manipulated using laser beams.
- Photonic Qubits: This approach leverages the quantum states of photons.
Each type has its advantages and drawbacks, largely dependent on the specific application and environmental conditions. They are also susceptible to decoherence, where external noise disturbs the state of the qubit, thus decreasing the effectiveness of quantum computation.
Qubit Types and Their Applications
The diversity of qubit types directly correlates to their range of applications. Here are a few predominant qubit types and how they are utilized:
- Superconducting Qubits
- Trapped Ion Qubits
- Topological Qubits
- Photonic Qubits
- Applications: Widely used in quantum processors, they are popular in labs like IBM and Google due to their relatively easier manufacturability and scalability.
- Applications: They maintain coherence over longer periods compared to superconducting qubits. This quality makes them ideal for precise quantum operations, and they are often used in research environments focusing on quantum algorithms.
- Applications: Their fault-tolerance property makes them suitable for more resilient quantum computation by protecting against certain types of errors from decoherence. Microsoft is actively pursuing research in this area.
- Applications: Excellent for quantum communication systems due to their compatibility with fiber optics. Techniques such as quantum key distribution benefit from this type.
Quantum bits are crucial to unlocking the full capabilities of quantum computation. Their unique characteristics allow for innovations that classical computing may not achieve. By understanding qubits better, reseacher and professionals can navigate the potential applications and implications of quantum technologies for the future.
Current State of Quantum Computing Technology
The current status of quantum computing technology is essential to understand how far this field has come and its potential future developments. As researchers and organizations invest significantly in quantum research, it has become critical to assess existing capabilities, the platforms leading this innovation, and their performance benchmarks. This exploration illuminates the framework within which quantum technologies can evolve, significantly impacting industries around the globe.
Leading Quantum Computing Platforms
Several platforms currently dominate the quantum computing landscape. Each platform presents different architectures and approaches tailored to specific use cases. Some notable platforms include:
- IBM Quantum Experience: This is a cloud-based platform offering access to quantum processors. It allows users to build and run quantum algorithms with notable ease. The IBM Qiskit framework enhances usability through an open-source software development kit for programming quantum computers.
- Google Quantum AI: Google has made headlines with its advances in quantum supremacy. The Sycamore processor showcased computational results that would take classical computers thousands of years. Google focuses on developing quantum algorithms that can outperform classical counterparts in certain tasks.
- Microsoft Azure Quantum: Microsoft aims for seamless integration of quantum solutions with traditional cloud services. The Azure platform provides users access to varied quantum hardware and software tools through its Quantum Development Kit. It promotes flexibility as developers can work across different quantum hardware setups.
- D-Wave Systems: Focused on quantum annealing, D-Wave's systems target specific optimization problems. Despite criticisms regarding practicality, D-Wave's innovation showcases the diverse approaches to quantum computing.
Each platform has its unique selling point, and understanding these differences is paramount for businesses seeking to leverage quantum computing in their operations.
Performance Benchmarks
Performance benchmarks play a crucial role in evaluating quantum systems. These benchmarks help assess the capacity of quantum computers against classical systems and among themselves. Key metrics to consider include:
- Quantum Volume: This metric considers qubit count, gate fidelity, and connectivity. A higher quantum volume signifies a more capable quantum computer. IBM introduced this concept to characterize the overall performance of its quantum systems.
- Gate Fidelity: This measures the accuracy of quantum gate operations. High fidelity is necessary for reliable computations. Error rates should ideally be low to ensure valid outcomes in quantum algorithms.
- Speed of Quantum Algorithm Execution: The time taken to run specific quantum algorithms reveals speed advantages over traditional computing. Certain algorithms show polynomial or exponential speed-ups under favorable conditions.
These benchmarks make it possible for developers and researchers to create more efficient algorithms, driving further advancements in the field.
"The assessment of quantum computer performance is not only about speed but also about reliability and accuracy, crucial for real-world applications."
By focusing on current platforms and their performance benchmarks, researchers and businesses can better appreciate where quantum computing stands today and the profound impact it may have on various sectors going forward.
Applications of Quantum Computing
The application of quantum computing is a vital topic within the broader narrative of its development and implementation. As businesses and researchers delve deeper into the potential of this technology, understanding its applications becomes crucial for grasping its implications across multiple sectors. Quantum computing stands to disrupt traditional computing paradigms, offering gains in speed and efficiency that classical computers cannot achieve.
The importance of exploring quantum applications lies not only in theoretical advancements but also in their practical implications. Technology enthusiasts and industry leaders must consider the benefits, limitations, and ethical dilemmas that arise from the integration of quantum capabilities into existing systems. Below, we outline some key areas where quantum computing promises significant advancements:
Quantum Computing in Cryptography
Quantum computing's intersection with cryptography is one of the most prominent applications. At its core, quantum computing can theoretically break many of the encryption schemes currently used to secure digital communication. For instance, Shor’s algorithm allows a quantum computer to factor large integers exponentially faster than the best-known classical algorithms. This advancement poses potential risks to encryption protocols like RSA, which are widely used for securing data.
However, quantum computing also gives rise to new cryptographic methods, such as quantum key distribution (QKD). QKD enables secure communication by leveraging the principles of quantum mechanics to detect eavesdroppers. The reliance on the inherent properties of quantum states ensures a level of security that classical methods cannot guarantee. This area of development will require businesses to adapt or potentially overhaul their security architectures in order to stay ahead.
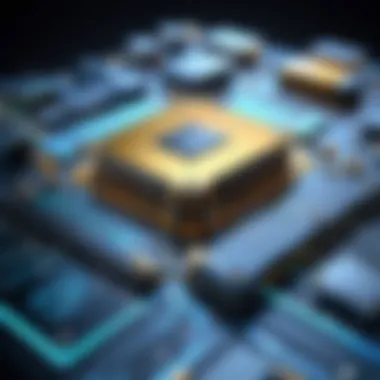
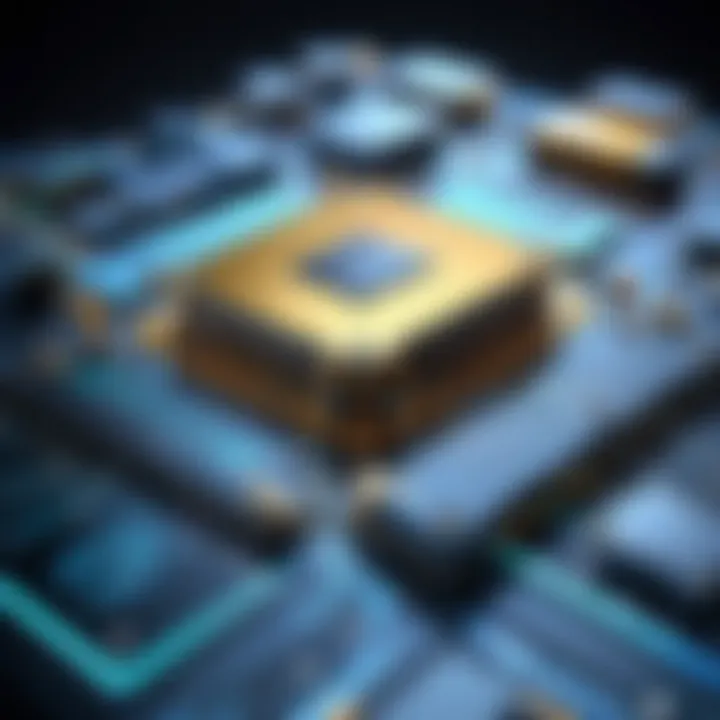
Optimizing Supply Chains with Quantum Solutions
The optimization of supply chains is another area ripe for exploration through quantum computing. Supply chain management involves complex logistics and a myriad of variables that can be challenging to compute efficiently with classical systems. Quantum algorithms can process large amounts of data more rapidly, leading to optimized routes, reduced costs, and improved operational efficiencies.
For instance, quantum algorithms can handle challenges like the traveling salesman problem more effectively, evaluating multiple scenarios at once to find the most efficient solutions. Companies leveraging quantum computing can anticipate demand fluctuations, optimize inventory management, and decrease lead times. Real-time analytics powered by quantum solutions could revolutionize how businesses interact with suppliers and customers alike, fostering a more agile response to market demands.
Advancements in Material Science
Material science represents another field where quantum computing can significantly alter research and development processes. Traditional methods of materials discovery can be slow and costly. Quantum simulations provide a method to model molecular interactions and predict the properties of new materials with high accuracy before they are synthesized in a laboratory.
Technologies such as quantum annealing are particularly adept at finding the optimal configurations of materials necessary for specific applications. For example, the discovery of new superconductors or lightweight composites could lead to highly efficient energy systems and innovative products. In this regard, quantum computing facilitates a more efficient experimental process, reducing time to market for new materials while minimizing resource waste.
Important Note: The transition to quantum-enabled material science applications will require interdisciplinary collaboration. Engagement among physicists, chemists, and engineers will be vital to unlocking the full potential of this technology.
In summary, the multifaceted applications of quantum computing underscore its potential to reshape entire industries. While challenges remain in terms of technical limitations and broader societal implications, the ongoing research in these fields highlights a transformative future. As quantum capabilities continue to evolve, their integration into practical applications will play a pivotal role in defining the technological landscape of the coming years.
Challenges Facing Quantum Computing
Quantum computing stands as a beacon of technological potential, yet it is met with several formidable challenges. Understanding these challenges is vital for stakeholders across various sectors. From researchers to enterprise-level executives, grasping the complexities of these impediments can foster informed decision-making and innovative solutions in the face of uncertainties.
Technical Limitations
One of the predominant technical limitations is the issue of scalability. Building quantum computers that can accommodate a larger number of qubits is a significant hurdle. As quantum systems increase in size, managing quantum coherence and entanglement becomes increasingly complex. The more qubits that are added, the more noise and instability can affect the calculations.
Another notable limitation is the fragility of quantum states. Qubits are susceptible to environmental interference, causing decoherence. This sensitivity makes it challenging to maintain stable quantum states long enough to complete computations. Recent advancements in superconducting qubits and trapped ions offer promising solutions but pose unique engineering difficulties.
The error rates of current quantum algorithms are another area of concern. The risk of error during quantum operations leads to incorrect outcomes, undermining the reliability of processes. Improvements in hardware and algorithm design are necessary to address these challenges.
"Quantum computing must overcome significant technical limitations to reach its full potential."
Some of the aspects concerning technical limitations include:
- Limited qubit connectivity: Not all qubits can communicate directly, hindering the optimal execution of algorithms.
- Noise and error rates: High error rates can result in inaccurate computations, raising the question of efficacy in practical applications.
- Resource constraints: It takes considerable resources and energy to maintain quantum systems, which can limit accessibility and deployment.
Quantum Error Correction
Quantum error correction serves as a crucial component for the advancement of quantum computing. The nature of quantum mechanics introduces unique challenges to error correction, requiring novel approaches distinct from classical error correction methods. The underlying principle of quantum error correction is to protect information stored in qubits from errors due to decoherence and operational noise.
To effectively implement quantum error correction, researchers have developed codes such as the Shor code and the surface code. These codes can safeguard quantum information by entangling multiple physical qubits to create a logical qubit. In essence, they create a redundancy that allows recovery from errors without measuring the quantum state directly, thus preserving the integrity of calculations.
There are several significant considerations when discussing quantum error correction:
- Complexity of Implementation: The mathematical underpinning is complex and calls for a deeper understanding of quantum mechanics.
- Resource Demand: Quantum error correction typically requires a large number of physical qubits to protect a single logical qubit.
- Performance Limits: Current quantum devices struggle to implement these error correction techniques fully due to limitations in qubit connectivity and operational fidelity.
In summary, while quantum computing exhibits immense potential, it faces substantial challenges rooted in technical limitations and error correction complexities. Addressing these elements is essential for driving innovation and realizing the practical applications of quantum computing.
The Future of Quantum Computing
The exploration of quantum computing's future holds immense significance in understanding its developing role in technology and society. As this domain progresses, it challenges traditional computing paradigms, leading to innovative solutions across diverse industries. The integration of quantum capabilities is poised to reshape expectations, especially in fields requiring extensive computational power. Furthermore, anticipating advancements helps stakeholders in businesses and academia prepare for the evolving landscape of tech.
Predicted Trends and Innovations
Quantum computing is projected to produce various trends that will influence many areas. In the short term, we can expect enhanced algorithms specifically optimized for quantum computers, allowing for solutions that surpass classical approaches in complexity and speed. Research is underway in quantum machine learning, which merges quantum computing with AI to improve data analysis efficiency and precision.
Other anticipated innovations include the development of more robust quantum hardware. Companies like IBM and Google are investing significant resources toward building fault-tolerant quantum systems. As these technologies mature, they will likely facilitate wider commercial applications. Moreover, as quantum infrastructure becomes more accessible, industries from finance to pharmaceuticals may begin integrating quantum computing into their operational practices.
- Improved quantum communication protocols will heighten security measures, making it harder for hackers to compromise sensitive data.
- Quantum simulation capabilities are expected to revolutionize drug discovery and material science, allowing researchers to model complex interactions at unprecedented speeds.
In summary, the predicted trends and innovations reveal a trajectory that is both exciting and full of potential, setting the stage for a new era in computing.
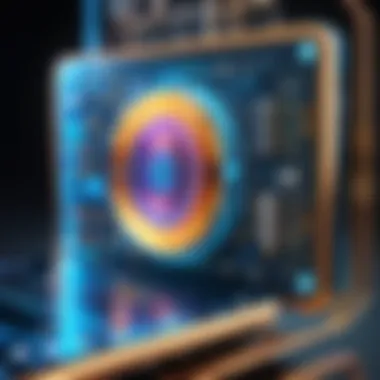
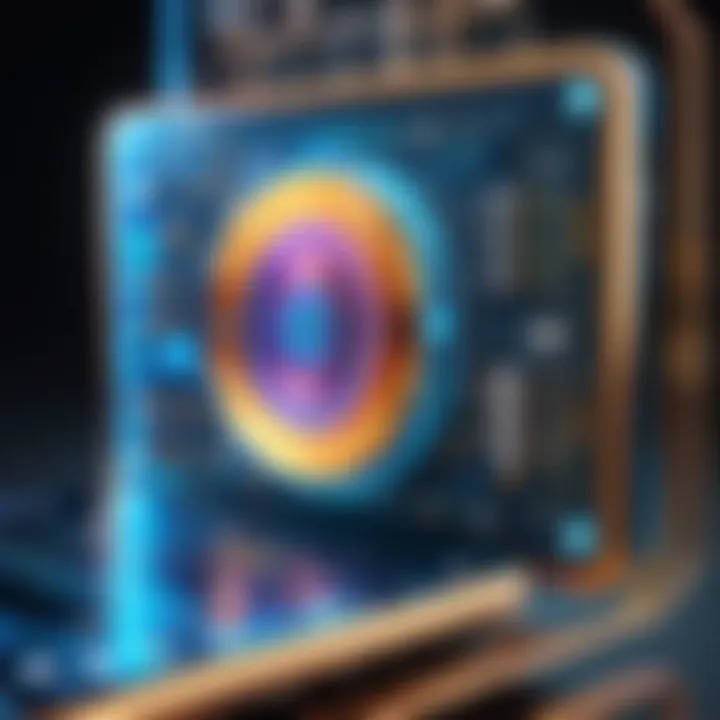
Integration with Classical Computing
Even as quantum computing is gaining traction, its synergy with classical computing methods can't be overlooked. The pathway to the future will likely involve a hybrid approach. This allows industries to leverage the strengths of both systems, maximizing computational efficiency and application versatility.
The integration will reflect in several ways:
- Complementary Functions: Quantum computers will tackle specific types of problems where they naturally excel, while classical computers will continue to manage tasks best suited to their architecture.
- Workflows Overlap: Developers will need to design workflows that efficiently exchange data between classical and quantum systems. This would ensure seamless operations in real-world scenarios.
Placing quantum capabilities alongside classical systems fosters a practical transition for businesses. By merging these technologies, organizations can harness the high-performance attributes of quantum computing while relying on existing classical systems for day-to-day operations. As a result, this integrated approach promises to yield more robust solutions and optimize resource utilization.
"The future of quantum computing is not just about the power of qubits but how we can coexist with and complement classical systems."
Ethical and Societal Implications
Exploring the ethical and societal implications of quantum computing is critical. This technology is set to revolutionize many sectors including finance, healthcare, and national security. However, it brings forth important questions about moral responsibility and societal changes.
Impacts on Security and Privacy
Quantum computing can significantly disrupt current security methods. Traditional encryption schemes, such as RSA and ECC, rely on the difficulty of certain mathematical problems. Quantum computers can solve these problems much faster than classical computers. For instance, Shor's algorithm allows for the factorization of large integers efficiently, posing a threat to current encryption standards.
This risk raises points on security and privacy vulnerabilities. Sensitive data, if not properly secured, could be easily accessed by unauthorized parties. Organizations and governments must prioritize developing quantum-safe cryptographic algorithms to protect data.
Here are some key considerations acting as guards against these risks:
- Transition to Post-Quantum Cryptography: Encourage exploring cryptographic methods that resist attacks from quantum computers.
- Policy Development: Formulate policies that regulate the use of quantum technology to protect privacy.
- Security Awareness: Educate organizations on the urgency of quantum threats and proper countermeasures.
"Quantum computing represents a paradigm shift in computational power; its implications for security must not be underestimated."
Creating a Quantum Workforce
The emergence of quantum computing requires a specialized workforce. The transition to this new technology demands skills that are scarce in today’s job market. Universities and training programs need to adapt quickly to prepare individuals for roles in quantum computing.
Key aspects to consider in workforce development include:
- Educational Programs: Create and enhance curricula in computer science, physics, and mathematics with a focus on quantum principles.
- Industry Collaboration: Encourage partnerships between businesses and educational institutions to foster talent and innovation.
- Continuous Learning: Promote the idea of lifelong learning in quantum technology to keep professionals updated on advancements.
Ending
In understanding quantum computing, it becomes clear that the implications reach far beyond mere theoretical exploration. This technology is on the threshold of reshaping industries, driving innovation, and addressing challenges that classical computing struggles to solve.
Summarizing Key Insights
Quantum computing, with its ability to perform complex calculations at unprecedented speeds, offers transformative potential across various domains. The following points summarize key insights:
- Speed and Efficiency: Quantum computers process information in ways that classical computers cannot, primarily through quantum bits or qubits. This allows for parallel processing, vastly improving computational efficiency.
- Revolutionizing Industries: Industries like healthcare, finance, and logistics stand to benefit immensely from quantum applications. For instance, in drug discovery, quantum computing can analyze molecular interactions more accurately and quickly than traditional methods.
- Challenges Ahead: Despite the promise, several challenges persist, including error rates in qubits and the difficulty of maintaining quantum states. Addressing these issues is crucial for realizing the full potential of quantum technology.
The Path Forward in Quantum Computing
Looking ahead, the landscape of quantum computing will likely evolve with significant advancements. Here are some considerations for the future as the technology matures:
- Integration with Classical Systems: A hybrid approach may emerge, combining the strengths of quantum and classical computing for optimal performance. This could enhance existing technologies while paving the way for new solutions.
- Workforce Development: Preparing the workforce for this transformation is vital. Educational programs need to incorporate quantum principles, ensuring a skilled labor market ready to address upcoming challenges.
- Regulatory Frameworks: As quantum technology finds practical applications, establishing regulatory frameworks will be essential. These frameworks will need to address security concerns, ethical considerations, and responsible usage.
"Quantum computing holds the promise of solving problems beyond the reach of classical computers, but its success depends on overcoming substantial technical and social challenges."
Importance of References
- Credibility: Citing reputable sources such as academic journals, articles, and expert opinions builds trust. When discussing complex topics like quantum computing, grounding arguments in established research is essential.
- Further Exploration: References give readers a pathway to explore further. Those interested can access additional resources to broaden their knowledge base. This is particularly important in a field that is so rapidly changing.
- Supporting Evidence: In any analytical piece, providing evidence is crucial. References offer the documentation needed to support specific claims or trends discussed within the text.
- Context: Given the historical context of quantum computing, references help frame discussions within the timeline of technological advancements, allowing readers to appreciate how the field has evolved.
- Diverse Perspectives: Including a variety of sources showcases different viewpoints. This diversity enriches the narrative by allowing readers to see various aspects of quantum computing, from theoretical frameworks to practical applications.
Considerations About References
- Accessibility: It is important to choose references that are easily accessible to the target audience. For example, links to pages like Wikipedia may provide straightforward explanations, while links to Britannica can enhance the scholarly authority of the information.
- Relevance: Ensure that all cited material is directly connected to the topics discussed. Irrelevant information may confuse rather than clarify.
- Recency: Quantum computing is a fast-evolving field. Citing the most recent studies or articles gives readers current insights that reflect today's realities, rather than outdated information.
- Diversity of Sources: Some readers may benefit from accessing technical forums like Reddit or professional networks such as Facebook, where they can find discussions and insights from practitioners in the field.
[Citing high-quality references enriches the analysis and enables readers to engage with the subject matter on a deeper level.]
In summary, the inclusion of a properly curated reference list at the end of the article is not merely a formality. It plays an essential role in enhancing credibility, providing avenues for further research, supporting claims, and encapsulating diverse viewpoints, all of which are crucial for a nuanced understanding of quantum computing.